Abstract
Comet 2I/Borisov is the first interstellar comet observed in the solar system, providing a unique opportunity to understand the physical conditions that prevailed in a distant unknown planetary system. Observations of the comet show that the CO/H2O ratio is higher than that observed in solar system comets at a heliocentric distance rh < 2.5 au. We aim to study the gas-phase coma of comet 2I/Borisov using a multifluid chemical-hydrodynamical model. The gas-phase model includes a host of chemical reactions, with the neutrals, ions, and electrons treated as three separate fluids. Energy exchange between the three fluids due to elastic and inelastic scattering and radiative losses are also considered. Our model results show that in the region of the coma beyond ∼100 km of the nucleus, e−−CO inelastic collisions leading to vibrational excitation of CO causes a loss of energy from the electron fluid. We find a high abundance of CO+ and HCO+ ions, and we show how these two ions affect the creation/destruction rates of other ions such as H2O+, H3O+, N-bearing ions, and large organic ions. We find that the presence of CO leads to a higher abundance of large organic ions and neutrals such as ,
, and CH3OCH3, as compared to a typical H2O-rich solar system comet. We conclude that the presence of a large amount of CO in the coma of comet 2I/Borisov, combined with a low production rate, affects the coma temperature profile and flux of major ionic species significantly.
Export citation and abstract BibTeX RIS
1. Introduction
Understanding the chemical evolution of prestellar cores to protoplanetary disks and, finally, the origin of the primordial compositions of planetary systems like ours is one of the fundamental questions in astrochemistry. Comets, made up of leftover material that formed the planets, are also the least-altered objects surviving from the protoplanetary disk that formed the solar system. Therefore they can preserve the signature of the physical processes and the chemical stratification that prevailed due to the spatial and temporal variation of volatiles in the disk. While comets in the solar system provide an understanding of the solar system formation, recent observation of the interstellar comet 2I/Borisov provides a unique opportunity to understand the physical conditions that prevailed in a distant unknown planetary system. However, it will be fascinating if future interstellar comets can be related to stars with known exoplanets; nevertheless, for now, the comet 2I/Borisov can be compared with solar system comets to derive valuable insights.
Comet 2I/Borisov (henceforth 2I) was discovered by G. Borisov on 2019 August 30. Initial characterization found a very high hyperbolic excess velocity of −32 km s−1, which was similar to the other known interstellar object 1I/'Oumuamua, and confirmed its interstellar origin (Jewitt & Luu 2019; Guzik et al. 2020). It was realized that comet 2I has a sizable nucleus of radius ∼0.5–1 km (Guzik et al. 2020; Jewitt et al. 2020). It crossed perihelion on 2019 December 8 UT at a heliocentric distance of 2.006 au (Jewitt et al. 2020). A large number of observations have been made to study the production rates of various volatiles. The water production rate is measured with a peak value of 10.7 ± 1.2 × 1026 mol s−1 (McKay et al. 2020; Xing et al. 2020; Opitom et al. 2021). It is found that the rate of increase of the water production rate pre-perihelion is greater than that in dynamically new comets, whereas the decrease post-perihelion is high compared to all previously observed comets (Xing et al. 2020). The CO production rate for comet 2I is found to be between 4.4 ± 0.7 × 1026 mol s−1 (Cordiner et al. 2020) and 10.7 ± 6.4 × 1026 mol s−1 (Bodewits et al. 2020). This makes comet 2I significantly CO rich and the CO/H2O ratio lies in the range 35%–105% (Cordiner et al. 2020), but can also be as high as 178% (Bodewits et al. 2020). Sublimation of the volatile CO ice can start from the cometary nucleus as far out as 120 au (Meech & Svoren 2004), and CO is an important driver of cometary activity at large heliocentric distances (Senay & Jewitt 1994; Womack et al. 2017). Water ice begins sublimating efficiently only at a heliocentric distance <2.5 au. There have been measurements of CO production exceeding water production outside 2.5 au (Ootsubo et al. 2012). However, the CO/H2O abundance ratio measured in 2I is substantially higher than that observed in other comets that came within a heliocentric distance of 2.5 au (Bodewits et al. 2020; Cordiner et al. 2020), which makes 2I a notable exception when compared with the solar system comets.
Besides H2O and CO, the production rates of HCN, CN, C2, and NH2 are also measured conclusively. The production rate of HCN is found to be 7.0 ± 1.1 × 1023 mol s−1, and the HCN/H2O ratio (0.06%–0.16%) is similar to that of the solar system comets (Cordiner et al. 2020), whereas the CN production rate is found to be (1.6 ± 0.5)−(9.5 ± 0.2) × 1024 mol s−1 (Fitzsimmons et al. 2019; Opitom et al. 2019; de León et al. 2020; Kareta et al. 2020; Aravind et al. 2021). Bannister et al. (2020) and Lin et al. (2020) detected C2 with a production rate of 1.1 × 1024 mol s−1 and 5.5 ± 0.4 × 1023 mol s−1, respectively, and a number of other authors provide an upper limit for the C2 production rate (Fitzsimmons et al. 2019; Opitom et al. 2019; de León et al. 2020; Kareta et al. 2020). It is found that in terms of the C2 production rate, 2I evolved from significantly C2 depleted to barely depleted (Bannister et al. 2020). NH2 was detected by Bannister et al. (2020) with a production rate of 4.8 × 1024 mol s−1. Measurements on the upper limits for C3 (Opitom et al. 2019), CH3OH, and CS (Cordiner et al. 2020) are also available.
In most of the solar system comets, H2O is the dominant constituent and can form up to ∼90% of the cometary volatile composition at distance <2.5 au (Combi et al. 2004). The next important parent volatiles in terms of abundance are CO and CO2. The average abundance of CO with respect to H2O is ∼5%, while it can vary over a range of 0.3%–41% (Dello Russo et al. 2016). The range of abundance for CO2 is a few up to ∼30% of H2O with a median value of 17% (Ootsubo et al. 2012). Trace amounts of other species such as NH3, H2S, HCN, and CH3OH are present, to name a few, whose abundance with respect to H2O is at the percent level or less (Mumma & Charnley 2011). At present, the number of parent volatile species, detected at least tentatively in comets, either remotely or in situ, stands at 72 (Rubin et al. 2019). Solar system comets exhibit chemical diversity in terms of their volatile composition, with the range of species abundances varying by a factor of a few up to several tens (Bockelée-Morvan 2011). Therefore it is pertinent to ask what chemical diversity is expected to be seen in the interstellar comet, and also how the abundances of secondary and tertiary species are affected when the production rate of CO is comparable to or more than that of water.
The application of combined hydrodynamical and chemical simulations to the coma helps in a quantitative understanding of the physics and chemistry occurring in the coma gas. The cometary coma model that we have used involves collision-dominated flow, a multifluid treatment of the coma gas, a chemical reaction network, and heating and cooling mechanisms. The model is described in Section 2 and the chemical network is also discussed. The production rates of volatiles and input conditions are specified in Section 3. In Sections 4 and 5, the model results and discussions are presented. Finally, in Section 6, the concluding remarks are made.
2. The Model
We provide a brief overview of the model in this section and a full description in Appendix A.1. In our model, we simulate the chemical and dynamical evolution of the coma of comet 2I. After the parent species sublimate from the nucleus, they undergo photochemical dissociation/ionization to form radicals, ions, and photoelectrons. All of the species in the coma undergo a host of gas-phase chemical reactions, which include collisional reactions between ions and neutrals, recombination reactions, and electron impact reactions. The rate constants for many of these reactions are a function of the gas temperature. Previous hydrodynamical models (Marconi & Mendis 1983; Körösmezey et al. 1987; Schmidt et al. 1988; Gombosi et al. 1986; Crifo 1991; Rodgers & Charnley 2002; Weiler2006, 2012; Hölscher 2015) found that in the coma, the gas temperature varies significantly with cometocentric distance. Thus, the inclusion of the dynamical evolution of the coma gas is necessary since it will have a considerable impact on the chemical evolution of the coma. The energy released due to the chemical reactions is not necessarily distributed uniformly among all of the species, resulting in different temperatures. Hence a multifluid approach, i.e., separating the neutral species, the ionic species, and the electrons, is used. Suprathermal species can trigger chemical reactions with high energy barriers, but Rodgers & Charnley (2005) showed that this effect may be important only in very active comets (Q ∼ 1031 mol s−1). Since we have done the modeling studies for comets up to an activity of ∼1029 mol s−1, we neglected suprathermal species. The expansion velocity for all species is assumed to be the same. There is coupling between the ions and electrons due to Coulomb interactions, resulting in charge neutrality. The assumption of a common velocity (plasma velocity) for the ion and electron fluids is thus reasonable. The electron temperature can attain very high values (∼104 K), causing the plasma velocity to become subsonic. To ensure a smooth transition through the sonic point, a separate numerical treatment would be required. This can be avoided if the plasma velocity is assumed to be the same as the velocity of the neutral species, so that all of the fluids move with a single bulk velocity (Rodgers & Charnley 2002; Weiler 2006, 2012; Hölscher 2015).
Our main focus is to study the coma chemistry using a large chemical network. Hence, we simplified the hydrodynamic equations by assuming the flow to be quasi-steady and spherically symmetric to make the calculations feasible. Since the timescale for changes in the comet's heliocentric distance can be much larger than the timescales for chemical reactions, the assumption of a quasi-steady state holds (Marconi & Mendis 1983). However, the shape of the nucleus will have an influence on the dynamical evolution of coma gases. Due to the scarcity of data on the shape of the nucleus, the assumption of spherical symmetry is more commonly used (Marconi & Mendis 1983; Rodgers & Charnley 2002; Weiler 2006, 2012; Hölscher 2015).
Apart from chemical reactions, we have considered the energy exchange between the fluids due to elastic and inelastic scattering processes. Elastic scattering can proceed via ion−neutral, electron−neutral and electron−ion collisions, and the resulting energy exchange is calculated as described in the Appendix A.4.2. For elastic electron−neutral scattering, collisions of electrons with H2O, CO, and CO2 are taken into account, using cross sections from Itikawa (2002, 2015) and Itikawa & Mason (2005). For electron−ion scattering we used the expression in Draine (1980) to calculate the heat transfer. Heat exchange due to the elastic collisions between neutrals and ions are considered within the reaction network as a special case of the ion−neutral reaction A + B+ → C + D+, with A ≡ C and B+ ≡ D+.
For inelastic electron−neutral scattering, cooling can occur due to both rotational and vibrational excitations of the neutral species by electrons. Solar system comets are predominantly water rich, therefore only cooling due to e−–H2O scattering is considered in the past models (Marconi & Mendis 1983; Körösmezey et al. 1987; Schmidt et al. 1988; Crifo 1991; Rodgers & Charnley 2002; Weiler 2006, 2012; Hölscher 2015). For rotational transitions, the cross section for e−–H2O scattering is up to four orders of magnitude larger than that of the next abundant molecule, CO, for low electron energies (Itikawa & Mason 2005; Itikawa 2015). Therefore, despite comet 2I being CO rich compared to other solar system comets, CO rotational excitations will have little cooling effect on the electron fluid compared to water. Thus we consider cooling due to rotational transitions for water only. At higher electron energies, vibrational excitation dominates over rotational excitation. The vibrational excitation cross sections due to collisions with electrons are of the same order of magnitude for both H2O and CO, and we considered vibrational excitation for both these species (Section A.4.3).
For water we used the analytical expressions for cooling rates of electrons by rotational and vibrational excitation of water molecules provided by Cravens & Korosmezey (1986). The cooling rates of electrons by CO vibrational excitations are calculated in the manner described by Waite & Cravens (1981). We also added a cooling mechanism by electronic excitation of CO due to electron impacts, and subsequent de-excitation (Table 4). This is directly included in the chemical reaction network due to the availability of Arrhenius coefficients (Schmidt et al. 1988). We have considered water−water collisions as a part of neutral−neutral inelastic collisions and used the empirical relation provided in Shimizu (1976). Other neutral−neutral collisions are neglected. The energy change due to inelastic electron−neutral and neutral−neutral collisions is discussed in the Appendix A.4.3.
The governing equations for the model are obtained from the conservation of number density, mass, momentum, and energy for each fluid (Weiler 2006; Hölscher 2015). These are a set of coupled first-order differential equations involving the species number densities, fluid temperatures, and outflow velocity. Numerical integration of these equations for a set of boundary conditions gives all of the physical and chemical properties of the coma gas as a function of the cometocentric distance. The numerical integration scheme is discussed in the Appendix A.2.
2.1. Initial Conditions
The initial gas velocity at the nuclear surface is assumed to be equal to the local sonic speed (Weiler 2006) given by

where γ is the adiabatic exponent, Rg is the specific gas constant, and T0 is the initial temperature of the coma gas. This temperature can be calculated from the surface ice temperature Ts . A Maxwellian velocity distribution of the outflowing gas is reached only after several molecular collisions, after which T0 and Ts are related as:

The initial temperature of all of the three fluids is set in this manner. In order to find the surface temperature, the energy balance equation is solved, and for a pure icy surface, this equation can be written as:

where F⊙ is the incident solar flux, which is scaled by the heliocentric distance rh
, Av
is the visual albedo, θ and ϕ are the local hour angle and the latitude, IR is the infrared emissivity, σB
is the Stefan−Boltzmann constant, H is the latent heat of sublimation of the ice, and Z(T) is the surface sublimation rate. If Q0,pri is the production rate of a parent volatile from the nucleus and r0 is the nuclear radius, then the number density of that volatile near the surface is

2.2. Chemical Network
To study the molecular complexity observed in the cometary coma, one needs chemical networks that contain reaction rate constants. The rate coefficients of bimolecular processes are expressed in the form of a so-called modified Arrhenius formula as follows:

where αj , βj , and γj are parameters obtained from literature and databases. For some reactions, different values of the parameters are needed for different temperature ranges. Rate coefficients for unmeasured ion−neutral reactions are calculated by Woon & Herbst (2009) using the Su−Chesnavich capture approach. Two separate formulae are used to compute the rate coefficients, one for lower temperatures and the other for higher temperatures, given by Equations (6) and (7), respectively.


The chemical reactions used in the model are taken from Schmidt et al. (1988), Weiler (2006), and Hölscher (2015), with necessary updates from the KIDA database (Wakelam et al. 2015, http://kida.astrophy.u-bordeaux.fr/). It also contains the parameters required to calculate the rate coefficients for the bimolecular reactions using Equation (5) and the ion−neutral reactions using Equations (6) and (7). The chemical network we have used contains more than 400 chemical species connected by about 4500 reactions. The reactions due to the electron impact excitation and radiative de-excitation of the electronic states of CO are taken from Schmidt et al. (1988). Reactions involving the metastable excited states of oxygen, carbon, and nitrogen are taken from Raghuram & Bhardwaj (2013) and Raghuram et al. (2020). The photolytic reaction rates are taken from Huebner & Mukherjee (2015) and Weiler (2006), appropriate for a quiet Sun. Optical depth effects are present in the coma, because of which the rates of the photochemical reactions decrease in the innermost regions. A detailed calculation of the photorates in the inner regions is done by dividing the solar UV radiation flux into various wavelength bins. The optical depth in each bin is then calculated using the photo cross sections and species number densities (details in A.5 of the Appendix). The binned photo cross sections are available at https://phidrates.space.swri.edu/#. The wavelength-dependent solar irradiance data set is available with the LASP Interactive Solar Irradiance Data Center (LISIRD), and we have used the flux derived from the FISM2 (0.1–190 nm) and NRLSSI2 (>190 nm) models (https://lasp.colorado.edu/lisird/). The flux is scaled to the heliocentric distance rh
of the comet as .
3. Input Parameters of 2I Coma Model
3.1. Volatile Production Rate of 2I
Figure 1 shows the production rates for observed volatiles for comet 2I; some of these are parent volatiles sublimating directly from the nucleus, while some are products of photochemical reactions. The molecular production rates for the different volatiles that are used as the initial inputs in our gas-phase model are given in Table 1. We modeled the coma at a heliocentric distance of 2.01 au post-perihelion; 2I was at this distance on 2019 December 15–16 and Cordiner et al. (2020) report on the production rates of several volatiles derived from observations made on these dates. The production rates of some of the cometary volatiles observed in comet 2I are not available for these dates. Hence, for these volatiles, we used the production rates derived from observations made on other dates or estimated them from some fitted relation. Cordiner et al. (2020) report QCO = (4.4 ± 0.7) × 1026 mol s−1 from the interferometric spectra and QCO = (5.0 ± 0.5) × 1026 mol s−1 using the autocorrelation (total power) spectra. Bodewits et al. (2020) report QCO = (6.4 ± 1.4) × 1026 mol s−1 for the observational epoch 2019 December 19–22. We use a conservative value of QCO = 5.0 × 1026 mol s−1, which is within the measured error limits. Bodewits et al. (2020) derived an empirical fitting for the H2O production rate. We have used this empirical relation to estimate around 2019 December 15.
Figure 1. Volatile production rates for interstellar comet 2I/Borisov. The uncertainties in measurements are shown by vertical error bars, while the downward pointing arrows indicate upper limits on the measurements. Reference: Fitzsimmons et al. (2019; CN, C2); Opitom et al. (2019; H2O, CN, C2, C3); Bannister et al. (2020; CN, C2, NH2); Bodewits et al. (2020; H2O, CO); Cordiner et al. (2020; CO, HCN, CH3OH, CS); de León et al. (2020; CN, C2); Lin et al. (2020; CN, C2, C3); Kareta et al. (2020; CN, C2); McKay et al. (2020; H2O); Xing et al. (2020; H2O); Aravind et al. (2021; CN, C2, C3); Opitom et al. (2021; H2O).
Download figure:
Standard image High-resolution imageTable 1. Production Rates of Volatile Species of Comet 2I Used as Model Inputs
Species | Production rate (mol s−1) |
---|---|
H2O | 5.4 × 1026 |
CO | 5.0 × 1026 |
HCN | 7.0 × 1023 |
CS2 a | 6.5 × 1023 |
CH3OH | 1.1 × 1025 |
C2H2 a | 3.0 × 1023 |
NH3 a | 5.0 × 1024 |
Note.
a Calculated from the product species abundance.Download table as: ASCIITypeset image
Cordiner et al. (2020) obtained the production rate of HCN and also derived upper limits on the production rates of CS and CH3OH. The upper limits on the relative abundances (with respect to H2O) of these species are consistent with values previously observed in solar system comets. We used the upper limit for CS derived by Cordiner et al. (2020) as our initial abundance for this species. The initial production rate of CH3OH is taken to be about 2% of H2O. This is a conservative value, which is roughly the average abundance of CH3OH with respect to H2O in solar system comets (Dello Russo et al. 2016). H2CO is an intermediate species in the formation of CH3OH by successive hydrogenation of CO. Thus H2CO is also expected to be present in the coma, although it is not detected. We obtained additional model runs with H2CO as a parent species. Lin et al. (2020) derived a power-law variation with heliocentric distance for the C2 production rate. Using this relation, and their measured mol s−1 at 2.145 au, we calculated
at 2.01 au for our model run. The production rate for NH2 was estimated by Bannister et al. (2020) from observations made in late 2020 November at a distance of 2.03 au pre-perihelion.
CS, C2, and NH2 are product species that are formed in the coma directly or indirectly by photodissociation of parent species. The CS radical is most likely to trace the parent molecule CS2 (Bockelée-Morvan et al. 2004). C2 is produced from several sources, including as a granddaughter species from the photodissociation of C2H2 (Combi & Fink 1997). The most likely candidate for the formation of NH2 in comets is the short-lived parent species NH3, and the abundance of NH2 is a direct measurement of the NH3 abundance (Wyckoff et al. 1989). We have converted the abundances of CS, C2, and NH2 into their parent species abundances from the branching ratios of the photolytic reactions (Fink & Disanti 1990).
3.2. Nuclear Size and Radiation Flux
Lee et al. (2020) used infrared imaging to infer an upper limit of 0.58 km for the nuclear radius rn . Jewitt et al. (2020) constrained the radius to be ≤0.5 km from measurements of the surface brightness profiles from the Hubble Space Telescope and an assumed albedo of 0.04. Guzik et al. (2020) estimated rn ∼ 1 km from the observed water production rate and an active fraction of 30%. We assumed a value of 1 km as the radius of the nucleus.
The FISM2 and NRLSSI2 flux models give the daily solar spectral irradiances. We collected the daily fluxes for the entire month of 2019 December from the LISIRD database. We then found the average spectral fluxes for 2019 December and scaled them to 2.01 au heliocentric distance. We used these scaled fluxes to derive the photorates as described in the Appendix A.5.
4. Results
We ran the model for two different input compositions: (1) the comet 2I composition, with production rates as given in Table 1 and (2) the 1P/Halley-type comet composition, with a net production rate of ∼1029 mol s−1, and the abundance percentages of volatiles with respect to H2O as given in Table 2. The nuclear size for the Halley-type comet was chosen to be 3 km, which is the average size for comet Halley used in the modeling studies by Marconi & Mendis (1986). We tested the model for a few other nuclear sizes for comet 2I, in the range ∼0.5–1 km, while keeping the production rate constant, and did not see any significant change in the results. In addition, we ran the model for the 2I-type comet composition, having relative abundances of volatiles similar to those of comet 2I but with a net production rate similar to that of a Halley-type comet (∼1029 mol s−1), to make a comparison of the abundance of complex ions (Section 5.3). The results of the model runs are shown in subsequent sections.
Table 2. Relative Abundance Percentage with Respect to Water, for Solar System Comet 1P/Halley (Bockelée-Morvan et al. 2004)
CO | CO2 | CH4 | C2H2 | C2H6 | CH3OH | H2CO | NH3 | HCN | H2S | CS2 |
---|---|---|---|---|---|---|---|---|---|---|
3.5 | 3 | 0.8 | 0.3 | 0.4 | 1.8 | 4 | 1.5 | 0.1 | 0.4 | 0.2 |
Download table as: ASCIITypeset image
4.1. Temperature Profile
The temperature profiles for the cases (1) and (2) mentioned above are shown in Figure 2, and Figure 3 shows some of the major heating/cooling rates per unit volume for the neutral and electron fluid. Our temperature profile for the Halley-type comet is qualitatively similar to the temperature profile for a Hyakutake-like comet obtained by Weiler (2006). The neutral temperature profiles shown in Figures 2(a) and 2(b) are relatively similar, with an initial dip and then a temperature inversion at a cometocentric distance of ∼200 km. However, the neutral fluid temperature falls to a much lower value (∼6 K) near inversion for comet 2I, as compared to an inversion temperature of ∼18 K for a Halley-type comet. In fact, at any cometocentric distance, the neutral temperature of the coma of the solar system comet is more than that of the interstellar comet. The main sources of heat for the neutral fluid in the coma are photodissociation and exothermic reactions. The photodissociation of H2O into OH and H produces 3.41 eV of energy, while CO photodissociates into C and O to produce 2.56 eV of energy (Huebner & Mukherjee 2015). Thus, the net rate of heat energy released per unit volume due to photodissociation is higher in the Halley-type comet because of a greater relative abundance of H2O. Besides, due to the larger production rate and higher density of the coma of the Halley-type comet, the net heating rate of the neutral fluid per unit volume from chemical reactions is greater.
Figure 2. Temperature profiles, i.e., variation of the fluid temperatures with cometocentric distance, for (a) interstellar comet 2I/Borisov and (b) a Halley-type comet. Tn , Te , and Ti denote the temperatures of the neutral, electron, and ion fluids, respectively.
Download figure:
Standard image High-resolution imageFigure 3. Variation of the heating and cooling rates per unit volume for the neutral and electron fluids for (a) interstellar comet 2I/Borisov and (b) a Halley-type comet. "Chem-n" and "Chem-e" denote the net energy gained due to chemical reactions by the neutral and electronic fluids, respectively. The energy lost by the electrons due to elastic scattering from H2O and CO molecules is denoted by "e-H2O elas" and "e-CO elas," respectively. Labels "e-H2O rot," "e-H2O vib," and "e-CO vib" denote the cooling of the electron fluid due to inelastic collisions with H2O (leading to rotational and vibrational excitation of H2O) and CO molecules (leading to vibrational excitation of CO).
Download figure:
Standard image High-resolution imageThe initial coupling between the electron and neutral temperatures is relatively weak for the comet 2I and they decouple at a shorter distance (∼20 km). The coupling is due to the inelastic collisions between the electrons and the water molecules, leading to rotational excitation of water molecules. The energy exchange rate due to collisions depends on the collision cross section as well as the number densities of the colliding species. The lower coma density for comet 2I implies that the frequency of such collisions is reduced. In addition, the dominant neutral species in the coma of comet Halley is H2O, while 2I has nearly equal production rates of both H2O and CO. This means that the relative number of H2O molecules available for rotational excitation is lesser in the interstellar comet. Therefore, the rate of energy exchange between the neutral and electron fluids is reduced in the innermost coma regions of 2I when compared to that of a Halley-type comet, as can be seen from Figure 3. The electron fluid loses less of its energy to the neutral fluid in comet 2I, and its temperature decouples closer to the nucleus. In the outer part of the coma, the electron temperature is high and vibrational excitation of neutral species by electrons becomes an important cooling mechanism for electrons. It is seen from Figure 3 that cooling of electrons due to vibrational excitation for CO is comparable to or larger than that for water for the coma of comet 2I. The energy loss rate per unit volume for electrons due to elastic scattering from the neutral species is several orders of magnitude less than the loss due to inelastic collisions (Figure 3).
4.2. Coma Chemistry
Comet 2I is unique in the sense that it contains H2O and CO in nearly equal proportions, which will have a considerable bearing on the ionic abundances. The variation of the flux of parent volatiles, electrons, and assorted ionic species as a function of cometocentric distance is shown in Figure 4. The flux is calculated as 4π r2 vn, where v is the velocity and n is the species number density. Plotting the flux removes the r−2 dependence, and rising/falling curve indicates that the species is getting created/destroyed by chemical reactions. H2O and CO together account for nearly 99% of the outgassing from the nucleus. Most of the parent species survive up to 105 km, consistent with an outflow velocity of ∼1 km s−1 and an average photolytic reaction rate of ≳ 10−5 s−1, leading to a nearly constant flux throughout the modeled region of the coma. NH3 and CS2 are exceptions, as they are destroyed faster by photolytic processes. We also note that since the number density is low, optical depth effects are nearly absent in the coma. Thus, the rates for the photoprocesses remain nearly constant throughout the coma.
Figure 4. Variation of flux as a function of cometocentric distance is shown for (a) parent volatiles and (b), (c), and (d) assorted ionic species and electrons in comet 2I.
Download figure:
Standard image High-resolution imageThe ions H3O+, HCO+, NH4 +, HCNH+, and CH3OH+ that are formed by the protonation (transfer of H+ ion) of parent species show an initial steep rise in the flux. As the density decreases on moving outwards, the timescale for the formation of these species becomes more than the dynamical timescale, and the curves tend to flatten. The flux of ions formed by photoionization or photodissociative ionization of parent species keeps rising in the outer coma as well, since the scale length for most of the parent species is ≳105 km. The dominant formation/destruction pathways for assorted ions, along with their relative reaction rates, are shown in Figures 5–7. The relative reaction rate is calculated by dividing the reaction rate for the creation/destruction process of a species by the total creation/destruction rate for that species. The relative reaction rate helps in understanding the contribution of each reaction toward the net creation or destruction of a species in different regions of the coma.
Figure 5. Major formation (solid lines) and destruction (dashed/dotted lines) pathways of (a) CO+ and (b) HCO+ for comet 2I.
Download figure:
Standard image High-resolution image4.2.1. CO+ and HCO+
The variation of the flux with cometocentric distance for the ions CO+ and HCO+ is shown in Figure 4(b), while Figure 5 shows the major creation and destruction mechanisms. The presence of a large amount of CO in comet 2I results in a high abundance of these two ions. The single most important mechanism that creates CO+ in the coma is the photoionization of CO, which accounts for almost all of its formation. CO+ is destroyed in the coma either through charge exchange or bimolecular reactions with neutral species. The destruction of CO+ by H2O accounts for up to 99% of the total loss rate of CO+ up to ∼5000 km. Beyond this distance, destruction by dissociative recombination increases on moving outwards, changing from ∼1% at 5000 km, to >20% in the outermost regions.
HCO+ forms when CO undergoes protonation, and also when H2O undergoes bimolecular reactions with ions such as CO+ and C+. The relative contribution to HCO+ formation by protonation reactions is slightly more than that by bimolecular reactions up to 500 km; the trend reverses beyond this distance. Photodissociative ionization of H2CO also creates HCO+ ions, and the rate for this process rises sharply beyond ∼1000 km. In the region of the coma >104 km, the relative contribution to the creation of HCO+ from ion−neutral reactions falls, and the photoprocess contributes to most of the HCO+ ion formation. HCO+ is destroyed in the coma by proton transfer reactions with the parent species H2O, CH3OH, and NH3, and by dissociative recombination. As in the case of CO+, the relative dissociative recombination reaction rates become important in the outer regions, accounting for up to 10% of the loss rate.
4.2.2. H2O+ and H3O+
The variation of the flux of H2O+ and H3O+ is shown in Figure 4(b) and their major formation and destruction pathways in Figure 6. The H2O+ ion, in addition to being produced by photoionization of H2O, is also produced when H2O participates in charge exchange reactions with ions present in the coma. CO+ is the most abundant ion with which H2O undergoes charge exchange, and this reaction accounts for 30%–35% of the net production of H2O+ within 100 km. H2O+ is mainly destroyed by proton transfer reactions with H2O and CO, and also with OH beyond ∼500 km. As with CO+ and HCO+, dissociative recombination reactions become steadily more important, and their contribution to the loss processes can rise up to 20%–25% in the outer regions.
Figure 6. Major formation (solid lines) and destruction (dashed/dotted lines) pathways of (a) H2O+ and (b) H3O+ for comet 2I.
Download figure:
Standard image High-resolution imageH3O+ is one of the more abundant ions in the coma, and it forms mostly by protonation of H2O by ions such as H2O+, HCO+, and OH+. Proton transfer reactions with the parent species CH3OH, NH3, and HCN account for most of the loss of H3O+ within ∼103 km. The relative rate for destruction of H3O+ by dissociative recombination is much more than that for other ions such as H2O+, HCO+, and CO+. In the outer regions beyond 104 km, this is the most efficient loss mechanism for H3O+.
4.2.3. N-bearing Ions
The variation of flux as a function of cometocentric distance for major N-bearing ionic species is shown in Figure 4(c) and the reaction pathways in Figure 7. Most of the in the coma is formed by photodissociative ionization of NH3, and a very small amount (∼2%) by charge exchange reactions between NH2 and abundant ions.
is formed by photoionization of NH3 and charge exchange of NH3 with ions (mainly CO+ and H2O+). Photoionization and charge exchange contribute nearly equally to
ion formation near the nucleus, but beyond 50 km, the charge exchange reaction rates begin to fall. The destruction of both
and
is primarily caused by H2O molecules.
reacts with H2O to form the protonated ion H3O+, and the relative rate for this reaction is 90%.
also reacts with H2O at slower rates to form
and NH4
+ ions.
reacts with H2O, and also with NH3 and OH to form NH4
+. Similar to previously discussed ions, dissociative recombination rates of
and
increase on moving outwards in the coma.
Figure 7. Major formation (solid lines) and destruction (dashed/dotted lines) pathways of (a) , (b)
and (c) NH4
+ for comet 2I.
Download figure:
Standard image High-resolution imageNH3 undergoes proton transfer reaction with many of the ions such as H3O+, H2O+, and HCO+ to form NH4
+. Apart from this, neutral species (mainly H2O and NH3) undergo bimolecular reaction with , and form NH4
+ by breaking the hydrogen bond. NH4
+ is mainly destroyed by dissociative recombination that proceeds via three channels.
HCN+ forms by photoionization of the parent molecule HCN and is destroyed by proton transfer and charge exchange reactions with the parent species CO and H2O. HCN undergoes protonation by ions such as H3O+, H2O+, and HCO+ to form HCNH+, and HCNH+ then successively causes protonation of neutral molecules H2O, NH3, and H2CO.
4.2.4. Complex Ions
The flux of assorted bigger organic ions are shown in Figure 4(d). CH3OH+ and H2COH+ are mainly formed by photoionization and photodissociative ionization of CH3OH, and small quantities of these ions are also formed when CH3OH respectively undergoes charge exchange and ion−neutral bimolecular reactions with the atomic ions H+, C+, O+, and N+. is formed by the protonation of CH3OH by the ions HCO+ and H3O+.
forms when a methyl cation transfer takes place from
to CH3OH. H2CO+ is a product of photochemical reactions undergone by CH3OH and H2CO.
The major loss mechanisms for CH3OH+, , and
are dissociative recombination reactions.
is also destroyed by CH3OH resulting in the formation of
; the relative loss rate for this reaction can go up to ∼25%, but falls off beyond 350 km. The major loss mechanism for both H2CO+ and H2COH+ are proton transfer reactions with H2O. Dissociative recombination reactions also destroy these two ions in the outer regions.
5. Discussions
Cometary observations over the years have established that H2O is the most abundant species in most of the solar system comets (Bockelée-Morvan et al. 2004). The focus of previous hydrodynamical and chemical models has been to study cometary comae dominated by H2O outgassing from the nucleus, though there have been few model studies for CO-dominated comae, for example, Ip & Mendis (1977), Crifo et al. (1999), and Raghuram et al. (2021). In this section, we discuss the differences seen in our modeled coma of comet 2I, as compared to those of water-dominated solar system comets.
5.1. Effect of CO Abundance on the Energy Exchange Rates
The electrons that are created due to photoionization and photodissociative ionization processes gain most of the excess energy released from the corresponding photochemical reaction because of their lighter mass. A dominant heat loss mechanism for electrons is inelastic scattering from neutral species, leading to the excitation of neutrals to the higher rotational and vibrational energy levels. H2O is a highly polar molecule and, in a water-dominated coma, inelastic scattering from this molecule is the primary reason for cooling of the electron fluid. Previous hydrodynamical models have only considered electron cooling due to water molecules because of a lesser abundance of other neutral species and a high e−−H2O collision cross section. In the case of the interstellar comet, where the CO abundance can be as high as or even higher than the H2O abundance, e−−CO inelastic collisions cannot be ignored. We have shown that in the high electron temperature and lower density region of the coma, e−−CO vibrational collisions are an important cooling mechanism. Electron-neutral temperature coupling exists up to some distance from the nucleus because the energetic photoelectrons efficiently lose their energy to the neutral fluid by rotationally exciting water molecules. This coupling that is observed in a water-dominated coma is also seen in the interstellar comet, though the coupling is weaker because of the reduced density, resulting from a lower production rate. The presence of a large amount of CO also reduces the net relative abundance of H2O, thereby reducing the cooling efficiency. If the CO abundance is increased even more, e.g., in comet C/2016 R2 (Biver et al. 2018; Wierzchos & Womack 2018; McKay et al. 2019), coupling may be lost altogether. However, it should be kept in mind that we have not considered rotational excitation of CO resulting from e−−CO inelastic collisions. Since the e−−CO collision cross section for rotational excitation is nearly four orders of magnitude less than that of e−−H2O collision, the net effect of including rotational excitation of CO may cause only a fractional change in the temperature profile of comet 2I.
5.2. Rates of Chemical Reactions and Ionic Composition of the Coma
Many types of chemical reactions occur in the coma, but two particularly important processes that drive ion chemistry in the coma in terms of creating new ions are photochemical reactions and proton transfer reactions. The dominant ions in the coma are the protonated parent species and the products of photoionization and photodissociative ionization of the parent molecules. The protonated molecules are more abundant within ∼100 km, while the photoproduct ion abundance takes over in the outer regions. The abundant ions H3O+ and HCO+, formed by protonation of H2O and CO, in turn cause protonation of other parent species to produce other ions, namely NH4
+, HCNH+, and . Hence, an important feature of the coma chemistry is a protonation"chain" whereby successive protonated species are created.
Ions in the coma undergo dissociative recombination reactions, which are dependent on the temperature of the electrons. The rates for these reactions are determined by Equation (5), where T is the electron temperature and, typically, the parameters βj ∼ −0.5 and γj = 0. Thus, these reaction rates decrease with an increase in the electron temperature and vice versa. From Figures 5–7, we see that all of the dissociative recombination reaction rates show an inversion at ∼30 km, and the relative loss of ions by these processes slows down sharply. This happens because there is an electron temperature inversion, followed by a sudden increase in the electron temperature at this distance. The primary mechanism by which electrons lose heat in the coma is due to inelastic collisions with the neutral species. The electron temperature decouples from the neutral temperature once the frequency of such collisions is reduced (Section 4.1). Subsequently, the heating rate of the electrons per unit volume due to chemical reactions becomes more than the cooling rate, resulting in the temperature inversion. On moving outward in the coma, the relative rates of the dissociative recombination reactions again increase, due to the continuous increase in the flux of photoelectrons. This inversion in the dissociative recombination rate is also seen in the Halley-type comet, though it occurs further out from the nucleus, at a distance of ∼1300 km, due to the delay in the electron fluid decoupling and temperature inversion. Besides, the relative reaction rates reduce at most by a factor of 2 in the Halley-type comet, whereas they can reduce by up to a factor of 4 in the interstellar comet. The reduction in the rate coefficients is compensated for by the enhanced electron flux, resulting in a lower net reduction for the Halley-type comet. The rates at which the chemical reactions occur in the coma depend on a host of factors such as the temperature dependence of the reaction rates, the availability of the reacting species and the optical depth effects (for photochemical reactions). In general, we see that the relative rates for the reactions involved in forming or destroying a particular species do not necessarily stay constant throughout. The dominant process by which a species is created or destroyed gets altered in different regions of the coma.
The production of H2O+ ion generally occurs by photoionization of H2O in water-dominated comets. However for comets with a large amount of CO like 2I/Borisov, a large amount of CO+ will be produced and charge exchange of H2O with CO+ is also an important formation mechanism of H2O+. A large amount of HCO+ is also present in our modeled coma of comet 2I because of a high CO and CO+ abundance. Such high quantities of HCO+ would not be seen in water-dominated comets because of a lesser amount of CO. HCO+ is an important ion that causes protonation of neutral species. The approximate contribution of HCO+ to the formation rate of H3O+, NH4
+, and is up to 30%–40%, ∼5%, and up to 20%, respectively.
The flux profiles of H2O+ and CO+ ions for comet 2I are nearly alike and this can be explained as follows. These two ions are primarily produced by photoionization at similar rates. In terms of loss processes, H2O+ is destroyed mainly by proton transfer reactions with H2O and CO, while CO+ is destroyed by charge exchange and ion−neutral reactions with H2O. The Arrhenius rate coefficients (Equation (5)) for the loss processes for H2O+ and CO+ are similar. The production and loss rates will also depend on the number densities of H2O and CO, which again, are almost alike.
5.3. Volatile Composition and Abundance of Organics
The presence of CH3OH is not conclusively found in the comet 2I; Cordiner et al. (2020) reported an upper limit of 4.4 × 1026 mol s−1 on the production rate of CH3OH. Solid state laboratory studies show that CH3OH can form in CO-rich ice at low temperature, by successive hydrogenation of CO, while H2CO forms as an intermediate product (Hiraoka et al. 1994; Watanabe & Kouchi 2002; Fuchs et al. 2009). The large amount of CO in the icy nucleus of comet 2I can produce sufficient quantities of CH3OH, but it was not conclusively detected, possibly due to lower activity. Since 2I shows similarity to other solar system comets in terms of composition of some of the volatiles, we have used the average value of CH3OH for solar system comets (Dello Russo et al. 2016) as the production rate in our model. We have not included other organic species such as CH4 and C2H6 as parent species in our model for comet 2I, as there is no report on their detection, though these species are generally observed in solar system comets.
To see which of the organic species abundances are affected when we have more CO outgassing from the nucleus, we ran the model for the compositions given in Tables 1 and 2 but for a higher production rate of ∼1029 mol s−1. Since hydrogenation of CO will form H2CO as an intermediate product, we also ran an additional model for the 2I-type comet composition by adding a relative H2CO abundance that is 4% of that of water, which is also the H2CO abundance in the Halley-type comet composition. Figure 8 shows the flux variation for some of the larger organic ions and neutrals for the 2I-type and Halley-type cometary compositions. The species shown in Figure 8(a) exhibit a higher abundance in the coma for the 2I-type comet as compared to the Halley-type comet, by ∼1 order of magnitude. This can be understood from the reaction pathways discussed in Section 4.2.4. forms when H3O+ and HCO+ ions cause protonation of CH3OH. There is a considerably higher abundance of HCO+ ions in the coma of the 2I-type comet as opposed to the Halley-type comet, which increases the net protonation reaction rate of CH3OH and results in a higher
abundance. An increased density of
will result in a higher abundance of
(formed by methyl cation transfer from
to CH3OH). Dissociative recombination of
then leads to the formation of CH3OCH3. The radiative association of H2O and HCO+ leads to the formation of
, and this ion then undergoes dissociative recombination to form HCOOH. We can see that a high HCO+ abundance (resulting from a high CO abundance) is key to the increased abundance of these species in a 2I-type comet composition. Thus, the presence of large quantities of CO will accelerate the formation of some of the organic ions and neutrals in the gas phase.
Figure 8. Flux of large organic ions and neutrals for 2I-type (solid lines) and Halley-type (dashed lines) compositions. The species show (a) a higher abundance for 2I-type composition and (b) a nearly equal abundance for both compositions.
Download figure:
Standard image High-resolution imageCH3OH undergoes photoionization to form CH3OH+ and neutral−neutral collision reactions to form CH2OH and CH3O. Since there is a nearly equal abundance of CH3OH in the 2I-type and Halley-type comet compositions, these product species also show near similar abundances, as seen in Figure 8(b). CH3O and CH2OH show a sharp fall in the outermost region as they are destroyed by H2O in this region. The energy barrier for the destruction reactions is high, and this barrier is crossed in the high-temperature region of the outer coma.
Figures 9(a) and (b) show the abundance of assorted species with and without H2CO added to the 2I-type comet composition. H2CO+ forms by photoionization of H2CO and photodissociative ionization of CH3OH, while protonation of H2CO produces H2COH+. H2CO also undergoes an ion−neutral reaction with to form
(protonated methyl formate), which successively forms HCOOCH3 by dissociative recombination. Thus in the presence of H2CO as a parent volatile, these species are seen in larger quantities in both the Halley-type and 2I-type comet compositions, as is evident from Figure 9(a). Besides, the abundance is higher in the 2I-type composition, as compared to the Halley-type composition. This is because of a higher availability of HCO+ and
ions in the 2I-type comet composition. HCO+ causes protonation of H2CO to form H2COH+, and
reacts with H2CO to form successive species, as discussed above. When H2CO is not considered as a parent species for the comet 2I-type composition, only a small amount of H2CO is formed in the coma by the photodissociation of CH3OH. This is not sufficient to produce a higher abundance of molecules such as HCOOCH3 as can be seen from Figure 9(b). Thus, we can say that if any organic molecule is present as a parent species in a comet, then the ions and neutrals produced from this molecule will have a high abundance in the coma. In addition, the abundance of these product species will be more in a comet where there is enhanced CO outgassing.
Figure 9. Flux of assorted species for 2I-type (solid lines) and Halley-type (dashed lines) compositions (a) with H2CO and (b) without H2CO in the 2I-type composition.
Download figure:
Standard image High-resolution image6. Conclusions
We used a multifluid combined chemical-hydrodynamical model to study the coma of the interstellar comet 2I/Borisov. This comet shows a CO/H2O ratio that is higher than that observed in solar system comets within 2.5 au, which alters the chemistry and dynamics of the gas-phase coma. From our model results, we conclude the following:
- 1.The coupling between the electron and neutral fluid temperatures in the coma exists only up to a short distance of ∼20 km from the nucleus. In the higher temperature region of the electron fluid, an important mechanism by which cooling of electrons occurs is due to inelastic collisions resulting in vibrational excitation of CO.
- 2.Protonated ions formed by H+ ion transfer to parent species show a high abundance up to ∼100 km. Beyond this distance, the fluxes of these ions tend to flatten, and ions formed by the photolysis of parent species become more abundant.
- 3.CO+ and HCO+ abundances are high due to the presence of a large amount of CO. Both of these ions have a considerable effect on the formation/destruction rates of other ions such as H2O+, H3O+, N-bearing ions, and large organic ions. HCO+ plays a significant role in causing the protonation of neutral species.
- 4.A high abundance of CO results in a higher abundance of many large organic ion and neutral species.
is formed due to the protonation of CH3OH by H3O+ and HCO+ ions. Due to the higher abundance of HCO+ ions, more
is formed, which is a precursor of other organic ions and neutrals.
This work gives an understanding of the coma composition of comet 2I/Borisov. Studies are already underway to correlate the compositions of solar system comets with those of protoplanetary disks (Drozdovskaya et al. 2019; Eistrup et al.2019), which will aid in discerning the primordial disk composition. The volatile composition of comet 2I/Borisov and its high CO/H2O ratio as compared to that of solar system comets provides insights into the prevailing conditions of its host system. A comparative study of the interstellar comet with solar system comets will help make a chemical inventory of comet 2I's natal disk, including possible organics that could be present.
Besides, it is argued that a considerable fraction of Oort cloud comets are from the protoplanetary disks of other stars (Levison et al. 2010). However, the properties of such objects that would set them apart from comets that formed in the Sun's protoplanetary disk is unclear. Comet 2I/Borisov exhibits many properties that are similar to the solar system comets, and this can provide clues for an understanding of Oort cloud comets that may have had origins outside the solar system.
We thank the anonymous referee for constructive comments which strengthened the paper. The authors thank Vikas Soni, Aravind K., Susarla Raghuram, and Shashikiran Ganesh for useful insights and discussions. The work done at the Physical Research Laboratory is supported by the Department of Space, Government of India.
Appendix
A.1. Differential Equations
The governing equations for the steady state, spherically symmetric gas-phase coma (Weiler 2006, 2012; Hölscher 2015) are as follows:




These equations represent the conservation of number density, mass, momentum, and energy, respectively, for each fluid. In the above equations, r is the cometocentric distance, n is the number density of any chemical species, v is the common velocity for all fluids, and kB is the Boltzmann constant. Variables ρ, T, γ, and μ are, respectively, the mass density, temperature, adiabatic exponent, and average molecular mass corresponding to each fluid. The source terms N, M, F, and Q represent the net rate per unit volume for the generation of chemical species, mass, momentum, and energy, respectively. The energy source term Q can be replaced by the thermal energy source term G, such that

The Equations (A1) to (A4) are rewritten as a set of coupled first-order differential equations in n, v, and T, as follows.

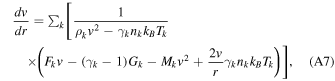

In the above equations, the subscript j indicates any particular chemical species and the subscript k = n, i, e stands for the three different fluids, namely neutral, ion, and electron, respectively. The terms nk and Nk are obtained by, respectively, summing over the number densities and number density source terms corresponding to the fluid k. The mass density ρk for any fluid is directly calculated from the number density as

where the summation is done over all of the species belonging to the fluid k, and m is the species molecular weight. The adiabatic exponent γk for a fluid is calculated as a weighted average of the adiabatic exponents γ for each species belonging to that fluid, with the species number densities as weights:

The value of γ for most species is listed in Schmidt et al.(1988).
A.2. Numerical Approach
The chemical reaction network (Section 2.2) contains rate coefficients that vary over many orders of magnitude. As a result, the differential equations used to model the coma are stiff. An implicit/semi-implicit integration method is required to solve the equations. Rosenbrock methods are reliable for solving higher-order stiff systems, and stable solutions can be arrived at by solving the Jacobian matrix. The first practical application of these methods was done by Kaps and Rentrop, so they are also known as Kaps−Rentrop methods. We have adopted the Fortran stiff integrator based on the Kaps−Rentrop algorithm as the numerical scheme (Press et al. 1992, Section 16.6).
A.3. Source Terms
The number density source term Nj for a species j is calculated by adding or subtracting together the rate coefficients of the reactions that contribute to the creation or destruction of that species.

Here, ν is the number of molecules of the species created in a particular reaction, is the reaction rate in cm−3 s−1, and the summation index
runs over all of those reactions in which the species j is a product or a reactant. Since the different fluids considered in this model have separate temperatures, the temperature-dependent collisional reaction rates are calculated at an effective temperature Teff (Flower 1985), such that

Tl and Tk are the fluid temperatures to which the reacting species having masses ml and mk belong. For reactions involving collisions with electrons, Teff ≈ Te , since the mass of the electron is much less than the mass of the other reacting species.
The total mass and momentum source terms are conserved, that is


Since these terms appear only as summations in Equation (A7), they need not be calculated individually for each fluid. The thermal energy source terms Gk for each fluid are sums of different components, which are discussed in the next section.
A.4. Thermal Energy Source Terms
The thermal energy source terms for the neutral, ion, and electron fluids, respectively, are defined as:



These components arise due to chemical reactions, the exchange of energy due to elastic and inelastic scattering processes, and radiative losses.
A.4.1. Chemical Reactions
The components ,
, and
denote the contribution to the thermal energy source terms of the neutral, ion, and electron fluids, respectively, arising due to chemical reactions. Production or loss of a chemical species results in the addition or removal of the kinetic energy of that species from the fluid that it belongs to. If a species j is created (or destroyed) by the reaction α, then its rate of increase (or decrease) of energy per unit volume is (Draine 1986):

Here, is the creation or destruction rate of species j by the reaction α, mj
is the mass,
v
j
is the mean velocity of species j,
w
j,α
is the mean velocity of the particles of species j emerging from or being used up by the reaction α, and ζj,α
is the random velocity of the particles of species j involved in the reaction α. Since the bulk velocity is assumed to be the same for all of the species,
, and only the internal energy
is required to compute Gj,α
. Chemical reactions also increase or decrease the energy of the system, depending on whether they are exothermic or endothermic, and this is the excess energy ΔE. The fraction of the excess energy received by the products is computed from energy−momentum conservation in the reference frame of the center of momentum of the reactants. Electrons that are created as products receive all of the excess energy, since their mass is negligible in comparison with that of the other species.
The thermal energy source terms for the different reaction types were computed by Draine (1986) and extended by Rodgers & Charnley (2002) and Weiler (2006). Table 3 lists the source terms , k = n, i, e for the species belonging, respectively, to the neutral, ion, and electron fluids, for the 15 generic types of reactions that are included in our network.
, when multiplied by the appropriate reaction rate
and summed over all of the chemical reactions in the network, gives the net component
, k = n, i, e.
Table 3. The Thermal Energy Source Terms , k = n, i, e Due to the Different Types of Chemical Reactions Included in the Model
Reaction type | Formula |
![]() |
![]() |
![]() |
---|---|---|---|---|
Photodissociation [1] | A + h ν → B + C | ΔE | 0 | 0 |
Photoionization [2] | A + h ν → A+ + e− | −Θn | Θn | ΔE |
Photodissociative ionization [2],[3] | A + h ν → B + C+ + e− |
![]() |
![]() | ΔE |
A + h ν → B + B' + C+ + e− |
![]() |
![]() | ΔE | |
Neutral−neutral [2],[3] | A + B → C + D | ΔE | 0 | 0 |
A + B → C + D + E | ΔE | 0 | 0 | |
Ion−neutral a [1],[3] | A + B+ → C + D+ |
![]() |
![]() | 0 |
A + B+ → C + ![]() |
![]() |
![]() | 0 | |
A + B+ → C + ![]() |
![]() |
![]() | 0 | |
Radiative association of neutrals [2] | A + B → C + h ν | −Θn | 0 | 0 |
Radiative association of ions & neutrals [2] | A + B+ → C+ + h ν | −Θn |
![]() | 0 |
Ionizative association [3] | A + B → C+ + e− | −2Θn | 2Θn | ΔE |
Radiative de-excitation [3] | A* → A + h ν | 0 | 0 | 0 |
Radiative recombination [1] | A+ + e− → A + h ν | Θi | −Θi | −Θe |
Dissociative recombination [1],[3] | A+ + e− → B + C | Θi + Θe + ΔE | −Θi | −Θe |
A+ + e− → B + C + D | Θi + Θe + ΔE | −Θi | −Θe | |
A+ + e− → B + C + D + E | Θi + Θe + ΔE | −Θi | −Θe | |
Electron impact ionization [2] | A + e− → A+ + e− + e− | −Θn | Θn | ΔE |
Dissociative electron impact ionization [3] | A + e− → B + C+ + e− + e− |
![]() |
![]() | ΔE |
A + e− → B + ![]() |
![]() |
![]() | ΔE | |
Electron impact dissociation [2] | A + e− → B + C | 0 | 0 | ΔE |
A + e− → B + C + D | 0 | 0 | ΔE | |
A + e− → B + C + D + E | 0 | 0 | ΔE | |
Electron impact excitation [3] | A + e− → A* + e− | 0 | 0 | ΔE |
Notes. Θk
is the mean kinetic energy of a particle belonging to the fluid k such that ,
, and Θe
= kB
Te
. ΔE is the mean exo/endo-thermicity of the chemical reaction.


References: [1] Draine (1986), [2] Rodgers & Charnley (2002), [3] Weiler (2006).
Download table as: ASCIITypeset image
A.4.2. Elastic Scattering
Elastic scattering occurs between each of the fluids, resulting in three types of scattering processes, namely ion−neutral, electron−neutral, and electron−ion, for which the rates of exchange of energy per unit volume are ,
, and
, respectively.
The ion−neutral elastic scattering is a special case of the ion−neutral reaction A + B+ → C + D+ given in Table 3, with mA = mC, mB = mD, and ΔE = 0. The most abundant neutral species in the coma are the parent molecules H2O, CO, and CO2, and the most abundant ions with which these do not undergo chemical reactions are H3O+, NH4
+, CH3OH+, , and HCNH+. Thus, ion−neutral elastic collisions can be modeled as "pseudo-reactions" of the kind

where there is only an exchange of energy and no change in the number densities of A and B. The rates for these reactions are calculated using Equation (5), with the Arrhenius coefficients αj ≈ 10−10 cm3 s−1, βj = 0.5, and γj = 0. The net change in energy for each collision is:

where mn
and mi
are the respective masses of the neutral and ionic species participating in the scattering process. is found by multiplying the energy change given by Equation (A20) with the collision rate per unit volume, and summing over all ion−neutral elastic collision reactions.
Similar to the case of ion−neutral scattering, the elastic scattering between electrons and neutrals is dominated by scattering from H2O, CO, and CO2 molecules, such that

where ,
, and
are the rates of exchange of energy per unit volume due to elastic scattering of electrons from H2O, CO, and CO2 molecules, respectively. The momentum transfer collision cross sections of electrons in these neutral gases has been compiled by Itikawa (2002, 2015) and Itikawa & Mason (2005), over a wide range of electron energies. To find the collision rate per unit volume of electrons with a neutral species, the relevant cross sections are averaged over a Maxwellian velocity distribution, and then multiplied by the number densities of electrons and the colliding neutral species. The average change in energy per collision
can be found from Equation (A20), by replacing the mass of the ion with the electron mass and Θi
with Θe
.
is then calculated by multiplying
with the corresponding collision rate and then summing over the three neutral species.
Elastic scattering between ions and electrons due to Coulomb interactions results in the following rate of energy change per unit volume (Draine 1980):
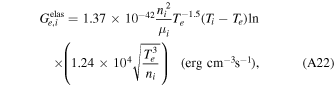
where ni is the total number density of all ions and μi is the mean ionic molecular mass.
A.4.3. Inelastic Scattering
For inelastic electron−neutral scattering, H2O and CO are the only neutral species that are considered. The per unit volume rate of cooling of the electron fluid due to these types of collisions is thus given by

Inelastic collisions of H2O molecules with electrons results in rotational and vibrational excitation of the molecules. The net cooling rate per unit volume of electrons due to inelastic scattering with H2O is:

The first term on the right is the cooling due to rotational excitation and the second and third terms are due to vibrational excitations. The analytical expressions for these were derived by Cravens & Korosmezey (1986) and are given below.


Terms and ne
are the number densities of water molecules and electrons, j = 1 corresponds to the vibrational transition (000) → (010), and j = 2 stands for the sum of the transitions (000) → (100) and (000) → (001). The values of a, b, Wj
, and Ij
in the above expressions are given by Cravens & Korosmezey (1986).
Vibrational and electronic transitions of CO due to inelastic collisions with electrons are considered to calculate the term . The rotational excitation of CO is ignored, because the cross sections for these transitions due to inelastic collisions with electrons is less than that of H2O by up to four orders of magnitude. The vibrational cooling rate per unit volume for 0 → j vibrational excitation for CO can be calculated in the manner given by Waite & Cravens (1981) for molecular hydrogen:

Here, nCO and ne
are CO and electron number densities, L(E, Tn
) is the loss function for vibrational excitation and de-excitation, is the electron thermal velocity, and fe
(E, Te
) is the normalized Maxwellian electron energy distribution function. The loss function for the transition 0 → j is

where Wj is the threshold for transition j and Tn is the neutral temperature. Term σj (E) is the energy-dependent cross section for transition j, taken from Itikawa (2015). The transitions j = 1, 2, 3 are considered; higher transitions are ignored because of reduced cross sections.
The reactions for the electronic excitation of CO by electron impact and its subsequent de-excitation are shown in Table 4. These reactions are included in the network, and the energy change is calculated as described in Section A.4.1.
Table 4. Reactions Showing the Excitation and De-excitation of the Electronic States of CO, Resulting in the Cooling of the Electron Fluid (Schmidt et al. 1988)
Electron impact excitation | Radiative de-excitation |
---|---|
CO + e− → CO(1Π) + e− | CO(1Π) → CO + h ν |
CO + e− → CO(3Π) + e− | CO(3Π) → CO + h ν |
CO + e− → CO(3Σ) + e− | CO(3Σ) → CO + h ν |
CO + e− → CO(3Δ) + e− | CO(3Σ) → CO(3Π)+ h ν |
CO(3Δ) → CO + h ν | |
CO(3Δ) → CO(3Π) + h ν |
Download table as: ASCIITypeset image
Neutral molecules with a permanent dipole moment can be excited to higher rotational states due to self-collisions. Energy can be lost from the neutral fluid (mainly H2O) due to infrared rotational emission, resulting in the cooling of the neutral fluid, such that

An empirical formula for the energy loss due to H2O–H2O collisions was given by Shimizu (1976):

Since optical depth effects cause radiation trapping in the inner regions of the coma, the radiation cooling rates are scaled by ; (Schmidt et al. 1988), where

Here, r0 is the nuclear radius, n is the number density of H2O, and σIR = 4 × 10−15 cm2 is the average infrared absorption cross section per molecule.
A.5. Photochemistry
Solar UV radiation causes photodissociation, photoionization, and photodissociative ionization of the gaseous cometary species. The absorption of the UV radiation in the coma causes attenuation of the UV flux. If ϕi,∞ is the unattenuated flux corresponding to the wavelength λi , then the UV flux at a cometocentric distance r is given by

where τi is the optical depth at wavelength λi , and can be calculated from the wavelength-dependent photochemical reaction cross sections and species number densities. For a cometary species denoted by j, if nj is its number density and σij,tot is its total photolytic cross section in λi , then the contribution of this species to the optical depth is:

The total optical depth at distance r can be obtained by summing over j, such that τi (r) = ∑j τij (r). Once the fluxes are known, the photolytic rate coefficient in the wavelength interval λi and λi + Δλi , and at a distance r can be found out using the relation

This can be approximated as ki (r) = σi Φi (r), where σi is the wavelength-averaged photo cross section in the ith bin that has a width of Δλi , and Φi (r) is the attenuated spectral photon flux integrated over the same wavelength bin. The total rate coefficient for any photolytic process can then be written as:
